- Home
- Assessments
- Bioregional Assessment Program
- Clarence-Moreton bioregion
- 2.6.2 Groundwater numerical modelling for the Clarence-Moreton bioregion
- 2.6.2.3 Model development
- 2.6.2.3.2 Hydrogeological conceptual model
The hydrostratigraphy and the hydrogeological conceptual model for the Clarence-Moreton bioregion have been described in detail in companion product 2.3 for the Clarence-Moreton bioregion (Raiber et al., 2016b). It describes the geological, hydrogeological, ecological and hydrological characteristics of the Richmond river basin, which was identified as the area within the Clarence-Moreton bioregion where coal seam gas (CSG) development is most likely to proceed in the foreseeable future. This section summarises the major characteristics of the conceptual model for the Richmond river basin and its implementation in a numerical groundwater model.
2.6.2.3.2.1 Hydrostratigraphy and relevant model layers
There are three major types of hydrostratigraphic units with distinct hydraulic properties in the Richmond river basin within the Clarence-Moreton bioregion:
- alluvial aquifer systems: in the Clarence-Moreton bioregion, these unconsolidated to semi-consolidated aquifers can be differentiated into upper catchment, mid-catchment and lower catchment based on geomorphological features and topographic gradient
- fractured igneous rocks: in the Richmond river basin, the fractured igneous rocks consist mostly of extrusive volcanic rocks such as the basalts of the Lamington Volcanics
- sedimentary bedrock: in the Richmond river basin, the sedimentary bedrock consists of a sequence of aquifers and aquitards with highly variable hydraulic properties. This aquifer–aquitard sequence and the vertical relationships between them are shown in Figure 8. As these units can be very heterogeneous, they can form an aquifer in a particular part of the bioregion (e.g. at the margin of the Clarence-Moreton Basin where the rocks tend to be composed of coarse-grained materials), but can also act as an aquitard elsewhere (e.g. further away from the basin margin and/or at greater depth where the permeability is typically lower). The spatial (horizontal and vertical) relationships and relative thicknesses of inferred aquifers and aquitards in the Richmond river basin are shown in Figure 9.
The lowermost units (Koukandowie Formation and below) are not included in the Richmond river basin groundwater model as they are not currently utilised for groundwater extraction due to their considerable depth, their inferred low yields and the high groundwater salinity of groundwater contained within Koukandowie Formation and the Gatton Sandstone (McJannet et al., 2015).
Source: Modified from companion product 2.3 for the Clarence-Moreton bioregion (Raiber et al., 2016b)
Groundwater model layers
The seven hydrostratigraphic units above the Koukandowie Formation were simplified and represented by six layers in the numerical groundwater model (Figure 8). Some of the major characteristics of these layers are described below. More detail on the geology and hydrogeology of these layers is available in companion products 1.1, 1.2 and 2.3 for the Clarence-Moreton bioregion (Rassam et al., 2014; Raiber et al., 2014; Raiber et al., 2016b).
- Layer 1 – Alluvium, Lamington Volcanics and unconfined parts of other units. The alluvium and the volcanics are the major aquifers targeted for groundwater extraction in the Clarence-Moreton bioregion. In the upper and mid catchment, the alluvial aquifer sequences are predominantly unconfined with a maximum thickness of 15 to 30 m. In the lower catchment, the alluvial aquifers can be up to 40 m thick and are more likely to be semi-confined. The Lamington Volcanics cover large surface areas in the northern part of the Richmond river basin, where they have a median thickness of 105 m and a maximum thickness of approximately 800 m. In order to reduce computation time and enhance model stability required by stochastic groundwater modelling, the alluvium, the Lamington Volcanics and the unconfined parts of other bedrock formations were merged into a single unit. This top unit represents the uppermost unconfined layer of the numerical groundwater model, while the other layers were considered as confined units.
- Layer 2 – Grafton Formation. The Grafton Formation consists of the Rapville Member and the Piora Member. As indicated by the three-dimensional geological model of the Clarence-Moreton Basin in companion product 2.3 for the Clarence-Moreton bioregion (Raiber et al., 2016b), the Grafton Formation (as defined by Doig and Stanmore (2012)) has a median thickness of approximately 150 m and a maximum thickness of approximately 500 m. It consists of an upper and a lower member (Rapville and Piora members, respectively) that have different hydraulic characteristics. The Piora Member, the lower member of the Grafton Formation, is composed of medium- to coarse-grained quartzose sandstone with an extensive clay matrix (Doig and Stanmore, 2012). While sometimes described as an aquifer, its reported low bore yields of about 0.3 L/second suggest that it is more likely to be an aquitard (Parsons Brinckerhoff, 2011). The Rapville Member, the upper member of the Grafton Formation, is composed of interbedded sandstone, siltstone and mudstone, and is considered to be an aquitard or aquiclude (Doig and Stanmore, 2012). There are not enough data to identify the boundary between the two members in the model domain. Hence, they were simulated as one layer.
- Layer 3 – Bungawalbin Member. The Bungawalbin Member, which is composed of mudstone and carbonaceous mudstone interbedded with fine-grained sandstone, has a median thickness of about 94 m. It has been described as an aquitard that prevents vertical groundwater leakage (Doig and Stanmore, 2012).
- Layer 4 – Kangaroo Creek Sandstone. The Kangaroo Creek Sandstone Member is an aquifer composed of quartzose sandstone and conglomerate (Doig and Stanmore, 2012). The estimated median thickness of the Kangaroo Creek Sandstone using the three-dimensional geological model is about 175 m and its maximum thickness is 370 m. Yields are mostly low (less than 1 L/second), except where fractures are intercepted (McKibbin and DLWC, 1995; Parsons Brinckerhoff, 2011). However, this assessment is based on limited data from the eastern part of the basin. Doig and Stanmore (2012) indicated that the Kangaroo Creek Sandstone has poor aquifer properties below a depth of 150 m.
- Layer 5 – Maclean Sandstone (Walloon Coal Measures). The Maclean Sandstone forms the upper part of the Walloon Coal Measures. It overlies the coal-bearing strata of the Walloon Coal Measures in the Richmond river basin (Figure 8). As indicated by the three-dimensional geological model in companion product 2.3 for the Clarence-Moreton bioregion (Raiber et al., 2016b), it has a median thickness of about 87 m and is described by Doig and Stanmore (2012) as an effective low-permeability top seal (aquitard) that extends over much of the basin and limits vertical leakage of gas from the coal seams to the surface.
- Layer 6 – Coal Seams (Walloon Coal Measures). The Walloon Coal Measures are typically considered as an aquitard in the Clarence-Moreton and the linked Surat basins. However, due to a spatially variable composition, their hydraulic characteristics may vary considerably. Numerous coal seams and carbonaceous coal shales have been intersected in the centre of the Logan sub-basin near Casino (Ingram and Robinson, 1996). The Richmond Seam (one of the youngest and thickest seams in the Walloon Coal Measures) is very extensive and can be correlated over tens of kilometres based on its distinct signal that can be identified in geophysical logs (Doig and Stanmore, 2012). The coal seams are separated by interburden (e.g. mudstone, siltstone, fine-grained sandstone and shale); recent well completion reports indicate that the net coal thickness is comparatively small relative to the overall thickness of this layer as detailed in companion product 1.2 for the Clarence-Moreton bioregion (Raiber et al., 2014). There was no differentiation between the interburden and the coal seams in this layer of the groundwater model due to the lack of data.
Groundwater model layer number is shown in brackets.
Source: Modified from companion product 2.3 for the Clarence-Moreton bioregion (Raiber et al., 2016b)
Hydrostratigraphic units not included in the groundwater model
The Koukandowie Formation and the Gatton Sandstone (both part of the Bundamba Group; refer to Figure 9), which underlie the Walloon Coal Measures, are poorly documented with no certainty as to whether they act as aquifers or aquitards throughout the Clarence-Moreton bioregion. However, the assessment of water quality in the Clarence-Moreton bioregion reported in companion product 1.5 for the Clarence-Moreton bioregion (McJannet et al., 2015), has shown that the Koukandowie Formation and the Gatton Sandstone have the highest groundwater salinity, with median electrical conductivities of 4750 and 5000 µS/cm, respectively. As they are low-yielding or partial aquifers in the Queensland part of the Clarence-Moreton bioregion (Raiber et al., 2016b), it seems more likely that these two hydrostratigraphic units are low-permeability aquifers or aquitards that are not suitable for groundwater extraction. As a result, the Koukandowie Formation and the Gatton Sandstone were excluded from the groundwater model.
2.6.2.3.2.2 Groundwater recharge and discharge
Groundwater recharge in the Clarence-Moreton bioregion occurs via different mechanisms, which include diffuse rainfall recharge and surface water recharge. The recharge assessment conducted as part of the Clarence-Moreton Bioregional Assessment for the Richmond river basin suggested that the entire extent of the Lamington Volcanics forms a preferential recharge area as presented in companion product 2.3 for the Clarence-Moreton bioregion (Raiber et al., 2016b). There is a high degree of hydraulic connection between the streams, and the alluvial and volcanic aquifers in the Richmond river basin with peak-level interaction in the headwaters of the alluvial systems that decreases downstream. In the headwaters of the alluvial valleys, rapid discharge occurs from the basalts to the thin and spatially restricted alluvial deposits where flow paths are short (often in the order of hundreds of metres) and residence times are small (in the order of days to months). Unlike the upper catchment where the alluvium is exclusively underlain by highly transmissive basalts of the Lamington Volcanics, the alluvium in the mid catchment is more likely to be underlain by sedimentary bedrock units, which may constitute aquifers or aquitards with substantially lower hydraulic conductivities than the Lamington Volcanics (hydraulic conductivity data are reported in companion product 2.1-2.2 for the Clarence-Moreton bioregion (Raiber et al., 2016a)).
Higher groundwater salinities in the alluvium of the lower Richmond river basin suggest that river recharge to the alluvium is likely to have a lower influence according to companion product 2.3 for the Clarence-Moreton bioregion (Raiber et al., 2016b) compared to that in the upper catchment. Diffuse recharge from precipitation seems to be the more dominant recharge process in the lower catchment. Unlike the upper and mid catchments, the lower Richmond River alluvia are underlain mostly by low-permeability sedimentary bedrock units such as the Grafton Formation (Figure 9). There is likely to be a smaller degree of hydraulic connection across the interface between the sedimentary bedrock and the alluvium (Raiber et al., 2016b). This suggests that the relative contribution of the sedimentary bedrock discharge to the overall alluvial water balance is small during normal climate conditions. In the eastern part of the Richmond river basin where different stratigraphic units thin and pinch out at the basin margin or are displaced by faults (Figure 9), upwelling of groundwater from the sedimentary bedrock into the shallow alluvial aquifers likely occurs. While the flow directions in the sedimentary bedrock of the Richmond river basin are poorly constrained by monitoring data, the bedrock topographic gradient derived from the three-dimensional geological model in companion product 2.3 for the Clarence-Moreton bioregion (Raiber et al., 2016b) suggests that groundwater flows towards the lowest point in the eastern part of the Richmond river basin, where it discharges to the alluvium and streams (Figure 9). However, while there are some elevated salinities in the alluvium that may indicate upward discharge of more-saline sedimentary bedrock groundwater, overall, most alluvial groundwater in areas where the alluvium overlies the down-gradient end of the sedimentary bedrock are fresh. This suggests that upwards leakage from the sedimentary bedrock may be overwhelmed by other sources of recharge to the alluvium, such as diffuse rainfall recharge or river recharge.
2.6.2.3.2.3 Aquifer connectivity
The hydraulic relationships between the different sedimentary bedrock units, the sedimentary bedrock and alluvial aquifers, and between the sedimentary bedrock and volcanic bedrock are much more difficult to determine compared to interactions between shallow aquifers. This is due to the complexity of the Clarence-Moreton Basin aquifer/aquitard system and the lack of groundwater observation bores in the sedimentary bedrock units. The current conceptual model for the Main Range Volcanics in Queensland assumes that there is only limited vertical connectivity across the interface between the basalts and the underlying sedimentary bedrock due to the presence of weathering horizons that have very low hydraulic conductivities as described in companion product 2.3 for the Clarence-Moreton bioregion (Raiber et al., 2016b).
Most sedimentary bedrock stratigraphic units in the Clarence-Moreton bioregion are considered as poor aquifers or aquitards according to companion product 2.3 for the Clarence-Moreton bioregion (Raiber et al., 2016b). If an aquitard is an ideal regional seal, then it should limit or prevent the vertical hydraulic connection between the overlying and underlying stratigraphic units. In the case of the Walloon Coal Measures of the central part of the Richmond river basin, Doig and Stanmore (2012) suggested that the high gas saturation levels are indicative of an effective seal that prevents or limits vertical gas leakage from the Walloon Coal Measures. This seal is thought to be the Maclean Sandstone, which is located between the coal seams of the Walloon Coal Measures and the Kangaroo Creek Sandstone Member. In addition to the Maclean Sandstone, there are two other stratigraphic units that are considered as aquitards (Bungawalbin Member and Rapville Member) separating the coal seams of the Walloon Coal Measures and the shallow alluvial and volcanic aquifers. While all aquitards appear to be present and continuous throughout the potential CSG development area west of Casino, their thickness and composition can vary so their role as regional seals may be compromised by the presence of geological structures (i.e. faults). Faults can cause compartmentalisation of the hydrostratigraphic units and act as horizontal barriers to flow (e.g. Moya et al., 2015; Smerdon and Turnadge, 2015). However, both faults and fractures can also act as vertical conduits.
Although several major geological structures have been recognised within the Richmond river basin where fault displacement is known to occur, their characteristics, such as depth, dip and hydraulic conductivity, are unknown. Faults were not represented in the Clarence-Moreton groundwater model due to the lack of the above mentioned information. The Metgasco groundwater model (Parsons Brinckerhoff, 2013) simulated the impact of a very simplified high-conductance vertical fault inside the development area on impact prediction; the modelling results suggest drawdown due to additional coal resource development (additional drawdown) is less than 2 m along the fault line within the Kangaroo Creek Sandstone that is overlying the Walloon Coal Measures. The impact of faults on predictions for shallow aquifers was not discussed in the Metgasco groundwater model. In addition, there are also likely to be unknown smaller geological structures, although further work and supporting data are required to identify them.
2.6.2.3.2.4 Extent of the groundwater model domain
The extents of the groundwater model domain were primarily based on an understanding of the geology and hydrogeology outlined in companion products 2.3 for the Clarence-Moreton bioregion (Raiber et al., 2016b). Overall, the groundwater model mostly follows the boundary of the Richmond river basin with deviations in some areas as follows (Figure 10):
- A small area of the Richmond river basin close to the Mount Barney intrusion was excluded from the northern part of the model domain. This was due to the very substantial geological complexity in this area and that it is more than 50 km away from the additional coal resource development area, hence, this deviation was deemed to have little or no impact on the groundwater modelling results. The only existing coal mines (operating or recently closed) within the Clarence-Moreton bioregion are located more than 120 km away from the potential CSG development area near Casino. In addition, the three-dimensional geological model of the Clarence-Moreton bioregion indicates that there is no hydraulic connection between the Bremer river basin (where the only operating coal mine is located) and the Richmond river basin, as multiple basement highs hydraulically separate the different depositional centres as described in companion product 2.3 for the Clarence-Moreton bioregion (Raiber et al., 2016b).
- In order to represent recharge into the Walloon Coal Measures, the western part of the groundwater model domain was extended to include the entire extent of the Walloon Coal Measures. This meant that part of the Clarence river basin west of the Richmond river basin was included in the groundwater model.
- It was outlined in companion products 1.5 (McJannet et al., 2015), 2.1-2.2 (Raiber et al., 2016a) and 2.3 for the Clarence-Moreton bioregion (Raiber et al., 2016b) that the lowermost units of the Clarence-Moreton Basin (i.e. Koukandowie Formation and below; Figure 8) are not currently utilised for groundwater extraction due to their considerable depth, their inferred low yields and the high groundwater salinity contained within Koukandowie Formation and the Gatton Sandstone. Consequently, the outcrop or subcrop areas of these older sedimentary bedrock units were excluded from the eastern and western parts of the groundwater model. Furthermore, due to their distant location from the additional coal resource development area, there are unlikely to be any impacts resulting from CSG development on water resources outside the extent of the Walloon Coal Measures.
Figure 10 Groundwater model extent
This map adopts the revised stratigraphic classification proposed by Doig and Stanmore (2012).
ACRD = additional coal resource development, PAE = preliminary assessment extent
Source: (i) Surface geology map of Australia 1:1,000,000; (ii) Geological Survey of NSW (In prep.); (iii) Queensland and NSW geological surveys
Data: Parsons Brinckerhoff (Dataset 1); NSW Trade and Investment (Dataset 2); Bioregional Assessment Programme (Dataset 3)
The potential area of CSG development (additional coal resource development) west and south of Casino is located centrally within the Richmond river basin (Figure 10). Based on results derived from previous modelling exercises in the Surat Basin where CSG development on a much larger scale was taking place (QWC, 2012; Moore et al., 2014), it was deemed that the current groundwater model boundaries were sufficiently far from the additional coal resource development, thus ensuring that all potential impacts associated with CSG development within the Richmond river basin are captured within the model domain with minimal boundary effects. This was further confirmed by an existing preliminary modelling study (Parsons Brinckerhoff, 2013) and a simple analytical element model specifically designed for the Clarence-Moreton groundwater model.
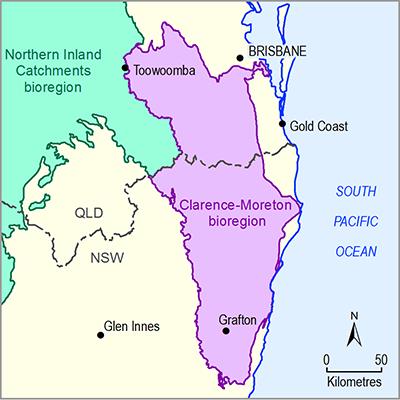
Product Finalisation date
- 2.6.2.1 Methods
- 2.6.2.2 Review of existing models
- 2.6.2.3 Model development
- 2.6.2.4 Boundary and initial conditions
- 2.6.2.5 Implementation of the coal resource development pathway
- 2.6.2.6 Parameterisation
- 2.6.2.7 Observations and predictions
- 2.6.2.8 Uncertainty analysis
- 2.6.2.9 Limitations and conclusions
- Citation
- Acknowledgements
- Contributors to the Technical Programme
- About this technical product