In the Clarence-Moreton bioregion, the only potential development that has been identified as likely within the time frames considered for the BA is Metgasco’s West Casino Gas Project (Raiber et al., 2014). There are no existing CSG developments in the Clarence-Moreton bioregion as outlined in Section 2.3.2. There is no hydraulic connection between the Richmond river basin and the Bremer river basin, where the only baseline coal mine (Jeebropilly Mine) is located. Due to lack of hydraulic connection between the baseline coal mine and the only additional coal resource development (Metgasco’s West Casino Gas Project in the Richmond river basin) (Section 2.3.2), it is scientifically justifiable to not model the baseline mine, and instead focus on West Casino Gas Project.
2.3.5.3.1 Coal seam gas operations
Hazards associated with CSG operations that are considered to be in scope for BA in the Clarence-Moreton bioregion are aggregated into four primary causal pathway groups. These four causal pathway groups represent a conceptual model of the chain of events that begins with an activity and ends with a potential impact on a groundwater or surface water dependent asset.
In this section, the four causal pathway groups associated with CSG activities are described to provide bioregion-specific context and show how different components of the hydrological system may be affected by potential CSG activities. The causal pathways are explained using a cross-section based on the three-dimensional geological model (presented in Section 2.3.2) through the area of potential CSG development in the Richmond river basin. The arrows on the diagrams highlight the potential causal pathways. The colours of hydrostratigraphic units correspond to the colours in the three-dimensional geological models and cross-sections of the Richmond river basin (Section 2.3.2).
2.3.5.3.1.1 ‘Subsurface depressurisation and dewatering’ causal pathway group
This group of causal pathways arises when coal mines and coal seam gas (CSG) operations intentionally dewater (in case of coal mines) and depressurise (in case of CSG operations) subsurface hydrostratigraphic units (such as coal seams and aquifers) to permit coal resource extraction. Dewatering applies to coal mines and will not be discussed further here. CSG operations involve groundwater extraction during the appraisal and production stages that aims to depressurise the Walloon Coal Measures to reduce hydrostatic pressure and facilitate gas release. In the Richmond river basin, the top of the Walloon Coal Measures is typically at a depth of about 400 to 700 m below surface. In addition to the intended flow of water within the seams towards the extraction wells, the declining water pressure results in pressure gradients that can potentially perturb the natural flow paths with potential impacts on the quantity and quality of groundwater (due to a change in inter-aquifer connectivity, Figure 37), as well as surface water (due to a change in surface water – groundwater interaction, Figure 37). In addition, water availability from overlying aquifers such as the Kangaroo Creek Sandstone that could lie within the depression cone (resulting from the abstraction) may be compromised (e.g. due to the reduced pressure). Those impacts may occur either on a point, local or regional scale and the presence and temporal variability of impacts is a function of aquifer composition, aquifer diffusivity and the presence or absence of aquitards and geological structures.
There is generally a declining likelihood of impact further away from the extraction well, both in the vertical and horizontal directions. That is, maximal impact is expected proximal to the extraction well in the Maclean Sandstone (upper part of the Walloon Coal Measures), which overlies the coal seams of the Walloon Coal Measures, and minimal impact in the alluvium and the Lamington Volcanics, which occur much farther away from the extraction well (Figure 37). As diffusivity decreases, the response of the groundwater system slows, which means that maximal drawdown takes longer to be attained. The final shape of the depression cone is a function of aquifer transmissivity, with highly transmissive aquifers having steeper cones where the drawdown is more confined near the extraction well.
The role of subsurface architecture and geological structure is important, especially the presence or absence of aquitards and faults, the location of faults relative to the extraction wells, fault displacements, the hydraulic role of faults as either barriers or conduits to flow and the composition and hydraulic properties of any fault-related infill material. For example, the Maclean Sandstone is a regional aquitard that can potentially prevent or limit the wider impacts of groundwater extraction from the Walloon Coal Measures, which may otherwise affect groundwater pressures in the Kangaroo Creek Sandstone. The Bungawalbin Member, also an aquitard, may further reduce or prevent propagation of impacts to shallow aquifers. Consequently, the integrity and continuity of such aquitards is paramount. Where faults or fractures are present, the pressure change can be transmitted much quicker and with potential impacts that may extend to the uppermost alluvial aquifer. However, this depends on the fault zone characteristics and the geometry of the geological compartments defined by the faults or fractures. Furthermore, it may be possible that prolonged depressurisation will reactivate a fault pathway, and thus create a pathway that was not active prior to the aquifer depressurisation.
If the extraction impacts extend all the way up to the alluvial aquifer, they might influence river recharge. Although this is likely to be of no or very minor consequence during normal and high-flow conditions (i.e. it is likely to be undetectable) and unlikely to have any impacts on major river systems such as the Richmond River due to the high flow rates described in Section 2.3.2, it could alter the low flow regime of smaller tributaries such as Shannon Brook with potential adverse impacts on water-dependent assets. Furthermore, declining watertable levels in the alluvial aquifer may influence the viability and function of groundwater-dependent ecosystems. The co-produced water, which varies in quantity and quality, when returned to the river after treatment has potential management implications that are discussed in the ‘Operational water management’ causal pathway.
The spatial and stratigraphic extent of drawdown caused by groundwater extraction from the coal seam can be assessed using numerical groundwater models. In the Clarence-Moreton bioregion, a numerical groundwater model is developed for the Richmond river basin, and the results of this model are reported in companion product 2.6.2 for the Clarence-Moreton bioregion (Cui et al., 2016).
Dewatering applies to coal mines and, given that no coal mines are being modelled in the baseline or CRDP in the Clarence-Moreton bioregion, it will not be discussed further in relation to the Clarence-Moreton bioregion.
CSG = coal seam gas, gw corresponds to groundwater; sw corresponds to surface water; TDS corresponds to total dissolved solids; TSS corresponds to total suspended solids
2.3.5.3.1.2 ‘Subsurface physical flow paths’ causal pathway group
One of the main activities of CSG operations is the drilling of wells that extend to the depth of the target coal seams or gas reservoirs (Figure 38). Wells are drilled during the exploration and appraisal phase to evaluate the potential commercial viability of the CSG resources, and during the production phase to depressurise the target coal seams and extract the identified gas resources. In addition, groundwater bores are installed to monitor potential impacts of CSG activities on groundwater levels or groundwater quality.
Maintaining well integrity throughout construction, operation and decommissioning phases is crucial to ensuring sustainable gas production and avoiding adverse environmental impacts. Throughout these stages of the CSG life cycle, preserving well integrity is required to prevent the inter-aquifer mixing of fluids (liquid or gas) and pressure changes, as well as preventing fluids escaping to the surface. Incomplete and/or compromised casing and seals (e.g. through degradation of the well casing or sealing material) could introduce preferential flow pathways along the well path. This newly introduced preferential pathway would have the potential to connect any two or more consecutive or non-consecutive geological layers up to land surface. The same causal pathway also exists for groundwater observation bores and other groundwater bores.
Potential impacts include the escape of gas from the coal seams to the overlying geological layers and potentially to the atmosphere (fugitive emissions). In addition, inter-aquifer mixing could potentially locally compromise the water quality of some aquifers such as the Kangaroo Creek Sandstone and the alluvial aquifer; adverse impacts on surface water systems are thought to be minimal due to the limited scale of the impact.
Leading practice is assumed for the construction and decommissioning of wells, and the impacts associated with compromised well integrity are likely to be of a local scale as the volume of water leakage is likely to be very small. Impacts resulting from a compromised well integrity are therefore limited to the vicinity (<1 km) of the compromised well although such impacts will continue until remedial actions are taken.
More general background on well integrity is provided by the Independent Expert Scientific Committee on Coal Seam Gas and Large Coal Mining Development (IESC, 2014a).
CSG = coal seam gas, TDS corresponds to total dissolved solids, TSS corresponds to total suspended solids
Hydraulic fracturing (or aquifer stimulation) is designed to increase the permeability within the target coal seams and enhance release and gas flow from the coal seams towards the well through these newly created fractures. The process of hydraulic fracturing during the production life-cycle stage of CSG operations involves high pressure injection of a ‘hydraulic fracturing fluid system’. These fluids typically consist of a slurry of water, proppant (a granular material that prevents the produced fractures from closing after the fracturing treatment) and chemical additives.
The water used for this process can either be sourced directly from a nearby river or from an alluvial aquifer; the potential associated impacts are similar to those discussed in the ‘Operational water management’ causal pathway group.
The intended impact of changing aquifer properties, when conducted properly, would be expected to be limited to the coal seams with a much smaller risk of impacting neighbouring aquifers or aquitards, such as the Maclean Sandstone. The lateral extent to which aquifer properties are changed diminishes further away from the well. No fracture monitoring data for the Richmond river basin are publicly available. However, Johnson et al. (2010) present some examples on the propagation of fractures from the Walloon Coal Measures in the linked Surat Basin. In these examples, in more than 50% of wells where an assessment was conducted, the fractures did not propagate above or below the perforated coal seam interval. Furthermore, where vertical propagation of fractures occurred, there were no reported cases where fractures propagated out of the coal measure and the maximum horizontal propagation that was recorded was 245 m.
Therefore, the scale of this impact is mainly dependent on the number of wells where this process is implemented. Due to the foreign materials added to the water used for hydraulic fracturing, the water quality of the perforated coal seams will be compromised, with a much smaller risk of contaminating neighbouring aquifers since it is a planned and well-monitored operation.
At the end of the fracturing procedure, hydraulic fracturing fluid is recovered from the coal seams (referred to as flowback) to desorb gas and permit its extraction to the surface. The recovered hydraulic fracturing fluid water has potential impacts similar to those discussed in the ‘Operational water management’ causal pathway group.
More details on the chemical composition of hydraulic fracturing fluids and their potential impacts on water-dependent assets are provided in the National Industrial Chemicals Notification and Assessment Scheme (NICNAS, in press). More general background on hydraulic fracturing is provided by the Independent Expert Scientific Committee on Coal Seam Gas and Large Coal Mining Development (IESC, 2014b).
gw corresponds to groundwater; sw corresponds to surface water; dashed line indicates that this process is unlikely to have any influence beyond the target coal seams
2.3.5.3.1.3 ‘Surface water drainage’ causal pathway group
The depressurisation of coal seams and overlying aquifers increases the effective pressures, which could lead to a consolidation of the various geological layers. This may exhibit itself as slight subsidence at the surface, which has the potential of altering the natural surface water drainage pathways (Figure 40) (Coffey Geotechnics, 2013; IESC, 2014c). Depending on the size of the CSG operations, the impacts might be of a small local or larger scale. There are currently no model predictions of subsidence available for the Clarence-Moreton bioregion. However, as the number of wells and projected water extraction volumes in the Richmond river basin are small compared to the CSG fields in Queensland, it is likely that subsidence is minor.
The construction of pipelines or other surface infrastructure related to CSG activities has the potential to permanently alter surface water flow paths. In addition, vegetation clearing during the construction phase may enhance soil erosion (e.g. following heavy rainfall on newly cleared areas), which can adversely affect the quality of surface water runoff.
Effects on surface water direction, volume and quality can have medium-term (5 to 10 years) to long-term (10 to 100 years) cumulative effects on watercourses within and downstream of the additional coal resource development area.
TDS corresponds to total dissolved solids; TSS corresponds to total suspended solids
2.3.5.3.1.4 ‘Operational water management’ causal pathway group
CSG operations require water during different stages of the life cycle (e.g. for the construction of exploration and appraisal wells and for the construction of development wells). However, significant volumes of water can also be produced during the gas production stage, and ultimately need to be disposed of (Figure 41). The water needed for CSG activities can be sourced either from surface water or groundwater systems. Direct extraction from surface water features such as rivers could affect their flow regime depending on the volume of extraction relative to streamflow. On the other hand, groundwater extraction directly influences groundwater availability as well as watertable levels that could potentially alter surface water – groundwater interactions; the latter can reduce baseflow or enhance river recharge in hydraulically connected river–aquifer systems.
The co-produced water, mentioned in the ‘Subsurface depressurisation and dewatering’ causal pathway group, may be disposed of via various methods. It can be transported off-site and outside the bioregion, thus eliminating any impacts in the bioregion. After treatment, it can be reinjected to other aquifers in the groundwater system. This can restore groundwater pressures and change the volume and timing of groundwater discharge to springs and watercourses in aquifer outcrop areas (although this would require a large volume of water and is therefore very unlikely to occur in the Clarence-Moreton bioregion based on the conceptual hydrogeological understanding presented in Section 2.3.2). Reinjection can also change aquifer chemical composition, with the extent of water quality changes being limited by local hydraulic properties of conductivity, storativity and time.
Co-produced water can be used for agriculture (such as irrigation) after adequate treatment and amendment; its post-treatment quality should be such that it does not adversely affect soil properties and the water resource.
Alternatively, treated water can be pumped back to the rivers, with potential impacts on their flow regime and water quality. Discharge of co-produced water to rivers and for irrigation can affect watertable levels and soil salt mobilisation along watercourses and near irrigation areas.
Finally, the co-produced water can be directed to storage ponds, with infiltration to the groundwater system posing a water quality risk. Furthermore, containment failure of the storage pond could potentially spread the risk over a much larger area.
Currently, it is not known which treatment options would be preferred if CSG development proceeds in the Richmond river basin. Any treatment and disposal will be subject to NSW Environment Protection Authority regulations.
More general background on co-produced water is provided by the Independent Expert Scientific Committee on Coal Seam Gas and Large Coal Mining Development (IESC, 2014d).
gw corresponds to groundwater; sw corresponds to surface water; TDS corresponds to total dissolved solids; TSS corresponds to total suspended solids
2.3.5.3.2 Outcomes and synthesis: linking causal pathways to potential impacts on water dependent assets for the coal resource development pathway
The integrated understanding of the geology, hydrogeology and surface water – groundwater interactions (Section 2.3.2) underpins the development of the numerical groundwater model reported in companion product 2.6.2 for the Clarence-Moreton bioregion (Cui et al., 2016). This model provides a quantitative assessment of the potential impacts of a CSG development in the Richmond river basin by providing estimates for potential drawdown and hence probabilities for exceeding certain thresholds that are identified by policy makers. This helps identify the direct impact that groundwater extraction has on economic assets and on groundwater-dependent ecosystems. Furthermore, the model estimates the potential changes in exchange fluxes between the surface and the groundwater systems, which will feed into the surface water models (reported in product 2.6.1 for the Clarence-Moreton bioregion (Gilfedder et al., 2016)) to calculate changes in flow indices that are critical in assessing potential impacts on surface water-dependent assets.
A qualitative impact assessment based on sound conceptual understanding of the system is warranted especially in the absence of numerical models (or in areas outside the model domain) and/or in cases where a full receptor impact analysis is not conducted. Knowledge of the geology, hydrogeology and surface water – groundwater interaction in the Richmond river basin provided the conceptual understanding for the identification of the major causal pathways, which were presented in Section 2.3.5.3.1. These causal pathways can support a qualitative assessment of the potential impacts on water-dependent economic, ecological and cultural assets. Hence, and based on this integrated conceptual understanding, an informed decision to classify various assets as either being potentially ‘impacted’ or ‘not impacted’ can be made.
The landscape classification introduced in Section 2.3.3 allowed the characterisation of the nature of water dependency among the diverse range of assets identified in the Clarence-Moreton bioregion. The causal pathways for CSG developments can be linked to the landscape classes identified in Section 2.3.3. Table 18 represents a conceptual framework that links various landscape classes with the identified four major causal pathway groups. Some of these landscape classes are exclusively related to groundwater, whereas others are related to surface water; some are related to both groundwater and surface water. Based on the conceptual understanding described in previous sections of this product that highlights how geology, hydrogeology, hydrology and ecology are related, a preliminary assessment was made on whether a particular landscape class can potentially be affected by a particular causal pathway or not. This allows the Assessment team to focus on landscape classes where the conceptual understanding of the Assessment team suggests that a causal pathway exists. Note that only the ’Subsurface depressurisation and dewatering’ causal pathway group will be modelled in the Clarence-Moreton bioregion. Impacts that occur via other pathways will be addressed merely as commentary.
The criteria that were adopted to undertake this qualitative assessment relates to the following aspects: (i) the spatial scale of potential impact associated with each causal pathway, for example, ‘Failure of well integrity’ and ‘Hydraulic fracturing’ are associated with local impacts, whereas ‘Subsurface depressurisation’ is associated with large-scale impacts, (ii) issues related to geology and hydrogeology such as topographic relief, recharge/discharge processes, and connection between various geological formations, (iii) tidal influence, (iv) the explicit location of the landscape class relative to the additional coal resource development with special emphasis on whether it is located upstream or downstream of the additional coal resource development, and (v) whether the dependency is on surface water, on groundwater, or both. In order to demonstrate this process, several contrasting examples from Table 18 that highlight the link between various landscape classes and causal pathways are described in more detail below. Note that some landscape classes may extend spatially across a large area. Therefore for some landscape classes, the last column of Table 18 can include asset examples that may or may not be affected by CSG developments; in such cases, if any asset is classified as potentially impacted, then the landscape is marked with a ‘Y’ (i.e. indicating that there is a causal pathway).
- Example 1 (‘Alluvium, high-energy upland, stream or river landscape class (landscape class number 4)): this example refers to the upper reaches (or headwaters) of rivers such as the Richmond River and its tributaries in the headwaters of the Richmond river basin. As this area is located far away (>30 km), and up-gradient from the potential CSG development area west of Casino, it was deemed that the ‘Failure of well integrity’, ‘Operational water management’ and ‘Hydraulic fracturing’ causal pathways and ‘Surface water drainage’ causal pathway group are not applicable. Unlike these causal pathways, which are likely to have more localised impacts, the regional-scale groundwater extraction from the Walloon Coal Measures to depressurise the coal seams could potentially affect landscape classes that are not within the vicinity of the potential CSG development area. Consequently, the ‘Subsurface depressurisation and dewatering’ causal pathway group has the greatest potential to affect wider areas. However, as shown in Section 2.3.2 of this product, recharge rates to the volcanic aquifers in the headwaters of the Richmond river basin and streamflow rates are very high, and there is likely to be only a limited connection between the basalts and the underlying sedimentary bedrock. Consequently, it is unlikely that there are any causal pathways that could link potential CSG developments to this landscape class. The groundwater model will test this conceptual understanding and determine whether such impacts are likely to occur or not.
- Example 2 (‘Alluvium, lowland, river’ landscape class (landscape class number 12)): examples of this landscape class include Shannon Brook, Myrtle Creek and Wilsons River. Both Myrtle Creek and Wilsons River are relatively far from the potential CSG development areas south and west of Casino (Section 2.3.2), therefore there would unlikely be any causal pathways between CSG activities and these streams. However, other streams in this category such as Shannon Brook flow through the area of potential CSG development west of Casino, and consequently, there are potential causal pathways that could link CSG developments with these streams.
- Example 3 (‘Estuarine, all terrain, and tidal-influenced river’ landscape class (landscape class number 20)): as outlined in Section 2.3.2, several of the streams within the Richmond river basin are influenced by tides. They are located in the eastern part of the Richmond river basin, but can extend more than 100 km inland due to the low topographic gradients. Even though they are downstream of the additional coal resource development area, there are unlikely to be any causal pathways that link CSG activities and these types of streams due to the tidal influence and the considerable distance from the potential CSG development area.
- Example 4 (‘Grassland GDE’ landscape class (landscape class number 28a)): this landscape class is restricted to the eastern part of the Richmond river basin near the coast. Due to the considerable distance from the potential CSG development area, there are unlikely to be any causal pathways that could link CSG developments and these landscape classes.
- Example 5 (‘Open forest GDE’ landscape class (landscape class number 31a): open forest groundwater-dependent ecosystems (GDEs) are widely distributed throughout the Richmond river basin, and are also present within or close to the potential CSG development area west of Casino. Due to this proximity to potential CSG development areas, there are potential causal pathways that could link the CSG developments with these water-dependent assets.
Table 18 Relationship between landscape classes and the four causal pathway groups in the Richmond river basin groundwater modelling domain
a‘Y’ denotes potential impact from the causal pathways that need to be assessed further; ‘N’ denotes unlikely impact. It is important to note that even if the initial assignment in this table suggests that a causal pathway does exist, the groundwater numerical model may indicate otherwise; note that the opposite scenario is also possible.
bExample assets listed are only examples of assets representative of each landscape class within the groundwater modelling zone that may or may not be in the potential impact zone for coal seam gas development.
It is worth highlighting that the initial assignment of Table 18 is preliminary; it will be tested with the aid of numerical models whose outcomes are reported in companion product 2.6.1 for the Clarence-Moreton bioregion (Gilfedder et al., 2016) and companion product 2.6.2 for the Clarence-Moreton bioregion (Cui et al., 2016). This means that even if the initial assignment in this table suggests that a causal pathway exists, the groundwater numerical model may indicate otherwise; the opposite scenario is also possible.
The conceptual framework presented in Table 18 allows identification of the level of risk associated with CSG development for each asset. A vulnerable asset assigned to a landscape class that has been identified as being potentially impacted through any of the four causal pathways (i.e. has a ‘Y’ in column 3 of Table 18) would be in a high-risk category. Conversely, an asset that is a member of a landscape class that is unlikely impacted through any of the four causal pathways (i.e. has an ‘N’ in column 3 of Table 18) would be in a low-risk category.
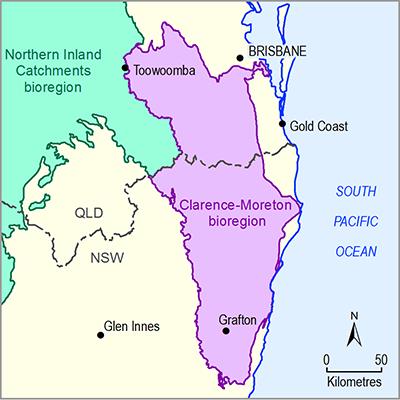