The hydrological investigation that supports the impact and risk analysis builds upon the surface water and groundwater modelling reported respectively in companion product 2.6.1 (Karim et al., 2018) and companion product 2.6.2 (Peeters et al., 2018) for the Galilee subregion. The modelling was designed to quantify potential changes in hydrology, such as changes in the magnitude and timing of streamflow or groundwater drawdown in an aquifer, caused by activities undertaken at multiple coal resource developments. This modelling approach enabled an assessment of the cumulative impacts of coal resource development at the regional scale of the Galilee assessment extent. The hydrological analysis has been used to define the zone of potential hydrological change for the Galilee subregion, outside of which potential impacts from the additional coal resource development are very unlikely (less than 5% chance of occurring). See Section 3.3.1 for more details, including the probability and exceedance threshold criteria used to determine the spatial extent of the zone of potential hydrological change.
3.2.3.1 Groundwater
The regional groundwater modelling for the BA for the Galilee subregion was undertaken using an analytic element model (AEM) implemented in TTim (Bakker, 2015). This was designed to simulate the change in drawdown at specific locations in the vicinity of the seven coal mines modelled in the CRDP, as well as the change in surface water – groundwater flux (Figure 10). The AEM was used to estimate spatially explicit probabilities of hydrological changes due to the proposed coal mining operations of the CRDP. Although BAs for other regions focus on potential hydrological changes for two futures (the baseline and the CRDP), there is no coal mining or CSG production in the Galilee subregion baseline as it is a greenfield basin for coal resource development. Hence, for the purposes of this assessment, the CRDP is defined by the seven coal mines comprising the additional coal resource development, and there is no separate reporting of baseline modelling results (Section 3.1.2.1).
As explained in companion product 2.6.2 for the Galilee subregion (Peeters et al., 2018), the main focus of the groundwater modelling is the hydrostratigraphic sequence of the Galilee Basin. This is conceptualised as an alternating sequence of aquifers and aquitards, which outcrop near the basin’s eastern boundary and dip gently westwards (Figure 11). The main regional Galilee Basin aquifer modelled here is the Clematis Group, although the deeper upper Permian coal measures (i.e. the main target for mining) are also classified as a partial aquifer (for modelling purposes the coal measures are aggregated into a single layer). The main aquitards simulated in the AEM are the Rewan Group, a thick aquitard separating the upper Permian coal measures and the Clematis Group, and the basal aquitard of the lowermost unit, the Joe Joe Group. Additionally, thin Cenozoic sediment layers are also incorporated into the model, as these unconformably overlie much of the Galilee Basin strata. The Cenozoic layers are conceptualised as an uppermost aquifer (Figure 11), which represents the highly permeable alluvial and colluvial sediments commonly associated with surface water systems such as the Belyando River and its tributaries. The underlying sediment layer is conceptualised as a lower permeability zone that represents a clay‑rich weathering profile (which formed during the Cenozoic). In total, the AEM has seven hydrostratigraphic layers, which are of infinite extent, constant thickness and uniform hydraulic properties across the entire modelling domain.
The geological units that belong to the stratigraphic sequence of the Galilee Basin occur sporadically at surface (outcrop) in a narrow north-trending zone adjacent to the proposed coal mines, and range from the basal (stratigraphic) Joe Joe Group to the upper unit of the Moolayember Formation. The Jurassic and Cretaceous units occur further west of the mines, and belong to the overlying (and geologically younger) Eromanga Basin. Most of the mapped area depicted here is covered by undifferentiated Cenozoic sediments, with Quaternary alluvium common along the rivers and streams. As explained in companion product 2.6.2 (Peeters et al., 2018), drawdown at the two nodes assigned to the Ronlow beds is not simulated directly in the analytic element model for the Galilee subregion, but is instead evaluated by proxy through drawdown in model layer 1 (alluvium and sediments).
ACRD = additional coal resource development, Fm = Formation
Data: Geological Survey of Queensland (Dataset 2); Bioregional Assessment Programme (Dataset 3, Dataset 4)
The limitations of the analytic element model mean that it is necessary to simplify the conceptual understanding of the hydrogeology in the central-eastern Galilee Basin depicted in (a) to the modelling conceptualisation shown in (b). In (b) aquifers are in dark orange, aquitards in dark grey. Green colours indicate stresses related to coal mining. Blue indicates surface water – groundwater interaction. Q is the mine dewatering pumping rate (Qmine). Δh is the change in groundwater level, which is set to ‑10 m in model layer 1 (Quaternary alluvium and Cenozoic sediments layer).
The hydrogeological units (layers) of the overlying Eromanga Basin sequence are not included in the AEM for the BA for the Galilee subregion. These layers are not present in the immediate vicinity of the modelled coal mines. Furthermore, they are stratigraphically separated from the Clematis Group by the low permeability aquitard of the Moolayember Formation, which is up to several hundred metres thick in this area. Consequently, any hydrological change in the Eromanga Basin layers would be much smaller than the change simulated in the Clematis Group. The decision not to specifically model the Eromanga Basin layers for this BA is justified by the Assessment team on the basis of sufficiently small hydrological changes predicted for the Clematis Group, and the known thickness and extent of the Moolayember Formation forming a substantial low permeability barrier to any potential drawdown propagation.
Groundwater modelling results reported in companion product 2.6.2 for the Galilee subregion (Peeters et al., 2018) focus on the three main aquifers simulated in the AEM, namely the:
- upper Cenozoic and Quaternary sediment layer
- Galilee Basin’s main regional aquifer of the Clematis Group
- upper Permian coal measures, which is the target geological unit for the coal mines.
For the purpose of the impact and risk analysis, most of the focus from the groundwater modelling is on the results from the uppermost Cenozoic sediment layer. This is because this is the hydrostratigraphic unit that mainly hosts the regional watertable within the area of proposed mining development. From a groundwater perspective, most of the groundwater-dependent landscape classes and ecological assets source their water requirements from the watertable, and hence hydrological changes simulated for this aquifer are of most concern for analysing impacts and risks for this BA.
Importantly though, there are some areas within the groundwater zone of potential hydrological change where the Cenozoic sediment layer does not exist. Rather, much older Triassic or Permian rocks outcrop at surface, and are here the likely host layers for the regional watertable (given the absence of Cenozoic sediments). In these areas, the main hydrogeological conceptualisation used in the Galilee subregion’s AEM may be considered invalid, and modelled drawdown results are very likely to be overestimated at the regional scale. In the areas of the modelling domain where Cenozoic sediments are absent, an alternative hydrogeological conceptualisation was presented in companion product 2.6.2 for the Galilee subregion (Peeters et al., 2018). The AEM outputs produced from this alternative conceptual model are expected to provide a more robust and accurate estimate of groundwater drawdown in areas where the regional watertable is not hosted in Cenozoic sediments (further discussed in Section 3.3). However, for the purposes of defining the zone of potential hydrological change (used as the basis for most of the Galilee impact and risk analysis), the more conservative conceptualisation (i.e. original version) which is applicable to the largest area of the model domain has been used.
There are also some economic assets (such as water access rights) and ecological assets (such as groundwater discharge springs) that may rely on access to groundwater from deeper confined aquifers in the Galilee subregion, such as the Clematis Group. For assessing potential impacts to these types of assets, the groundwater drawdown simulated for the relevant deeper confined aquifer layer must be used. Impacts to some important springs in the Galilee subregion, as well as economic groundwater assets, are discussed further in Section 3.4 and Section 3.5 (respectively).
The simulation of coal mining in the Galilee subregion assumes that the near-surface Quaternary alluvium and Cenozoic sediment cover (i.e. the uppermost aquifer modelled in the AEM) is completely removed during mining operations and that the mines act as a drain for the local Cenozoic aquifer system throughout the simulation period. This means that in the near-surface aquifer there is no post-mining recovery of drawdown simulated in the AEM. Consequently, should the Cenozoic cover sediments be partially restored as part of mine rehabilitation works before 2102, the time of maximum drawdown will be earlier than simulated. However, should the Cenozoic cover not be restored or only restored after 2102, a new dynamic equilibrium will be established between local aquifer recharge and mine void discharge. The extent of this dynamic drawdown equilibrium will likely be smaller than the extent simulated in this BA as the compensation of drawdown by recharge is not included in the AEM. However, the actual physical extent of the near-surface aquifer is a more substantial constraint on the extent of drawdown in it than the way the mine rehabilitation is implemented in the model. As explained in Section 2.6.2.9 of companion product 2.6.2 (Peeters et al., 2018) for the Galilee subregion, simulating the uppermost aquifer as a continuous groundwater system across the entire modelled domain adopts a very precautionary approach. This is because the actual extent of the upper aquifer as it exists in the central-eastern Galilee subregion covers a much smaller area than what is simulated in the model domain of the AEM. Consequently, the potential extent of drawdown derived from the regional modelling is likely to be overestimated.
Groundwater modelling results were generated at specified nodes across the modelling domain (Figure 10). Hydrological response variables representing the maximum drawdown and the year of maximum drawdown under the CRDP were defined for summarising the model results. Drawdown from the groundwater model nodes was spatially interpolated to obtain valid posterior distributions at all assessment units across the modelling domain. A Delaunay Triangulation was generated in the R package ‘tripack’ (Renka et al., 2015). For each assessment unit in the subregion (Section 3.2.4.1), the quantiles of maximum drawdown at the nodes of the triangle enclosing the assessment unit were linearly interpolated to the new location. A forward-backward cubed-root transform was applied during the interpolation to improve performance over potentially non-linear surfaces.
Section 3.3.1.1 describes how the groundwater modelling results have been used to define the groundwater zone of potential hydrological change, which focuses the remaining impact and risk analysis on areas where potential groundwater impacts may occur, and dismisses areas where impacts related to drawdown are very unlikely (less than 5% chance of occurring).
3.2.3.2 Surface water
Surface water modelling for the Galilee subregion was undertaken using the Australian Water Resources Assessment landscape model (AWRA-L). Details of the application of this model to the Galilee subregion are reported in companion product 2.6.1 (Karim et al., 2018). No river modelling was carried out because the rivers in the subregion are unregulated and their streamflow characteristics can be simulated solely by using a rainfall-runoff model (see companion submethodology M06 (as listed in Table 1) for surface water modelling (Viney, 2016)). This means that streamflow can be predicted by accumulating output from the spatially explicit streamflow model AWRA-L. The proposed coal mining development in the Galilee subregion will affect regional surface water hydrology directly through disruption of surface water drainage systems and some aspects of operational water management, and indirectly through changes in surface water – groundwater fluxes in response to mine dewatering. Consequently, the surface water modelling was designed to specifically assess these types of causal pathways.
Surface water model results for eight hydrological response variables were reported at 61 model nodes across the Galilee subregion and some adjacent river basins (Figure 12). These hydrological response variables were selected to characterise impacts of coal resource development, and were considered representative of flow characteristics that are important for assessing impacts to ecological and economic assets. Most of the model nodes are on streams in the Belyando river basin, which is a headwater catchment of the larger Burdekin river basin (Figure 12). In order to carry out the impact and risk analysis, results from these model nodes needed to be interpolated to specific stream reaches (i.e. stream links upstream or downstream of the model nodes). Interpolating these changes from nodes to links is an important step in analysing the changes in surface water across the entire assessment extent.
The process for interpolating hydrological response variable data from model nodes to stream links is shown schematically in Figure 13 and Figure 14. In addition, Table 7 provides information on some of the codes used in these schematic diagrams. The schematics include a number of stream links with no model nodes (dashed lines). Although it was not possible to generate model results for these stream reaches, they were important links for extrapolating results across the wider Galilee stream network. For example, the junctions of non-modelled streams with the modelled stream network may correspond to significant changes in streamflow and hence represent limits to interpolation from the nearest upstream or downstream model node.
As a starting principle, interpolations were not initially undertaken for stream links that contain inflows proximal to the coal mines. For example, the Carmichael River above node 14, the reach upstream of node 18 on Bully Creek, and the reach from a point upstream of node 5 to downstream of node 6 on Lagoon Creek – Sandy Creek are all proximal to one or more large open-cut mine pits and mine surface infrastructure. However, during the hydrological impact analysis (as outlined in Section 3.3), the Assessment team undertook more detailed evaluation of the results for the three hydrological response variables that are the focus of Section 3.3.3 (i.e. zero-flow days, high-flow days and annual flow volume). This involved further careful assessment of every interpolated reach in the stream network, and their adjoining node data both upstream and downstream. This work particularly focused on those reaches mentioned above where extrapolation was initially not done due to proximity to the coal mines. Working systematically through the data for the three hydrological response variables of interest, expert hydrological judgement was used to update the initial extrapolation schematic for some reaches that had previously not had node data assigned. This process resulted in an improved node-to-reach mapping that substantially increased the length of stream network for which the node data could be reliably interpolated. The results of the interpolations are presented in the series of maps showing changes in zero-flow days, high-flow days and annual flow volumes in Section 3.3.3.
Section 3.3.1.2 describes how the surface water modelling results were used to define the surface water zone of potential hydrological change.
The main focus of the hydrological modelling in BAs was on the maximum predicted change between the baseline and the CRDP for the surface water and groundwater hydrological response variables. As described in companion product 2.6.1 (Karim et al., 2018) and companion product 2.6.2 (Peeters et al., 2018) for the Galilee subregion, these maximum hydrological changes are primarily modelled to occur during and post-mining to the end of the 90-year simulation period (2102). However, the BA modelling approach did not examine hydrological changes after 2102, nor factor the medium- to long-term effectiveness of mine rehabilitation efforts. Additionally, post-mine closure legacy issues were not incorporated in the modelling including the potential impacts of any remaining open-cut mine pits on groundwater flow systems.
Figure 12 Surface water model nodes in the Galilee subregion
Probabilistic model outputs for eight hydrological response variables were estimated at the surface water nodes shown here, and reported in companion product 2.6.1 for the Galilee subregion (Karim et al., 2018). Four of these eight hydrological response variables characterise low streamflow, namely zero-flow days, low-flow days, low-flow spells, and length of the longest low-flow spell. Another two hydrological response variables characterise high streamflow (daily flow rate at the 99th percentile and high-flow days), and the remaining two characterise flow volume and variability (annual flow and interquartile range).
ACRD = additional coal resource development
Data: Bioregional Assessment Programme (Dataset 4, Dataset 5, Dataset 6, Dataset 7)
The red arrows that occur at some nodes denote the direction of surface water flow along the stream network, and also bound the interpolation source for discrete links in the stream network to specific model nodes (e.g. the segment of North Creek between the red arrow at node 15 and the red arrow at location X9* is assigned the model outputs generated at node 45). In contrast, the straight red lines only occur on stream links between model nodes (commonly where a smaller non-modelled tributary joins the modelled stream network), although they also bound the interpolation source for the link to a specific node. Further information about the locations on this diagram denoted by an ‘X*’ is in Table 7. Model node 29 at the top of this node – link schematic occurs at the start of Lake Dalrymple.
The red arrows that occur at some nodes denote the direction of surface water flow along the stream network, and also bound the interpolation source for discrete links in the stream network to specific model nodes (e.g. the segment of Native Companion Creek between the red arrow at node 36 and the red arrow at node 37 is assigned the model outputs generated at node 37). In contrast, the straight red lines only occur on stream links between model nodes (commonly where a smaller non-modelled tributary joins the modelled stream network), although they also bound the interpolation source for the link to a specific node. Further information about the locations on this diagram denoted by an ‘X*’ is in Table 7.
Table 7 Explanation of codes used in Galilee surface water model node to stream link interpolation schematics
This table explains the codes used for various small streams and point locations on the surface water interpolation schematics, shown in Figure 13 and Figure 14. The symbols X1 to X7 (inclusive), as well as X16, are shown in Figure 14. The symbols X8 to X15 (inclusive) are depicted in Figure 13.
3.2.3.3 Applying remotely sensed data
Several components of the impact and risk analysis have applied remotely sensed data (e.g. satellite imagery from Landsat) and derivative products such as tasselled cap wetness (Section 3.2.3.3.1) and the normalised difference vegetation index to aid in the assessment (Section 3.2.3.3.2). In particular, these tools have helped to evaluate the potential groundwater contribution (baseflow) to the minor stream network (non-modelled streams) in the zone of potential hydrological change, as well as assisting in the interpretation of the hydrological processes that support several distinct areas of the ‘Springs’ landscape group within the zone of potential hydrological change. The general remote sensing workflow used in this assessment involved:
- geographic overlay analysis, which involved overlaying the remote sensing datasets (using GIS tools and applications) with other types of geographic data, such as the stream network and digital elevation model data, within the zone of potential hydrological change
- assessment of distinctive spatial and temporal trends in the tasselled cap wetness and normalised difference vegetation index datasets within the zone, for example, correlating stream segments (or spring areas) with vegetation greenness and detectable surface water signatures (particularly in drought periods)
- qualitative evaluation of the non-modelled stream network within the zone to assess the level of groundwater dependency. As explained further in Section 3.3.1.2.2, this analysis was used to classify which parts of the non-modelled stream network in the groundwater zone of potential hydrological change may be potentially impacted due to additional coal resource development (although the magnitude of impact cannot be quantified as these groundwater-dependent streams were not modelled).
The specific application of these remotely sensed datasets and tools to improve understanding of the nature of surface water – groundwater interaction is detailed in Section 3.3.1.2.2. Further information about the ‘Springs’ landscape group is in Section 3.4.3, with specific spring-related ecological assets discussed in Section 3.5.2.
The remotely sensed data analysis used for this BA was undertaken in collaboration with researchers from the Digital Earth Australia team (Geoscience Australia, 2017). Digital Earth Australia (DEA) is an analysis platform for satellite imagery and other earth observation data that are based on the Australian Geoscience Data Cube (Lewis et al., 2017). DEA holds an archive of 30 years of corrected and processed earth observation data, and provides tools to interact with the data through the Australian National Computational Infrastructure (NCI) high performance computing environments. Published DEA products based on remotely sensed data include Water Observations from Space (WOfS) and the Inter-Tidal Extents Model (ITEM), a map of Australia’s intertidal zone (Mueller et al., 2016; Sagar et al., 2017).
3.2.3.3.1 Tasselled cap wetness
The tasselled cap wetness in the landscape summary output is produced using DEA. Landsat surface reflectance data at 25 m resolution with information in the red, green, blue, near infra-red and short-wave infra-red spectral bands are retrieved from the DEA archive using a spatial query for the area of interest. Clouds and areas of terrain shadow are masked from the surface reflectance data. A tasselled cap transformation is performed on each of the surface reflectance bands to produce a per-pixel ‘wetness’ value. This method is based on the tasselled cap transformation of Crist (1985), but only uses the component of transformed surface reflectance in the ‘wetness’ direction to identify the presence of water and wet vegetation.
Areas of water and wet vegetation are highlighted where pixels exceed a specified wetness threshold. Pixels exceeding the wetness threshold are counted through the temporal image archive. The wetness in the landscape summary is then presented as the percentage of scenes where the pixel has contained water or wet vegetation through time. Selecting a spatial transect of interest (e.g. a line that follows a river, or crosses a wetland or spring) allows for retrieval of the wetness threshold data for each image pixel along the transect line. Stacking the pixel information with respect to time (given the 30-year temporal coverage of DEA) can be used to create a Hovmöller plot, which allows for analysis of wetness (or other relevant variables, such as rainfall) along the transect line (or at particular points) through time. Examples of Hovmöller plots for selected transects in the zone of potential hydrological change are presented and assessed in Section 3.5.2.
3.2.3.3.2 Normalised difference vegetation index
The normalised difference vegetation index (NDVI) was initially developed in the late 1970s (Tucker, 1979). The NDVI provides a consistent metric of vegetation greenness for terrestrial vegetation (i.e. vegetation where there is no water present beneath the canopy within the pixel). Hovmöller plots of vegetation greenness provide a valuable way of characterising how persistent vegetation is, and whether it has an evergreen habit, or is responding to annual or multi-decadal patterns of rainfall. The Hovmöller plots presented in Section 3.5.2 also provide information about spatial and temporal variations in NDVI for selected areas within the zone of potential hydrological change in the Galilee subregion.
3.2.3.4 Representing predictive uncertainty
The models used in the BA for the Galilee subregion were run many hundreds or thousands of times and produced a large number of predictions of groundwater drawdown and streamflow characteristics rather than a single number. This results in a range or distribution of predictions, which are typically reported as probabilities – the percent chance of something occurring (Figure 15). This approach allowed an assessment of the likelihood of exceeding a given magnitude of change, and has underpinned the analysis of the risk posed to landscape classes and water-dependent assets due to coal resource development.
An important point to note here is that the probabilities reported in the BAs are conditional probabilities, that is, they are conditional on the various assumptions and limitations that have underpinned the development of the probabilistic models. The concept of conditional probabilities is critical to the uncertainty propagation methodology used in BAs (see companion submethodology M09 (Peeters et al., 2016)), as demonstrated through the emphasis on the qualitative uncertainty analysis reported in several products for the Galilee subregion, including companion product 2.6.1 (Karim et al., 2018) and companion product 2.6.2 (Peeters et al., 2018). Within these hydrological modelling products there are detailed descriptions and analyses of the key assumptions and limitations incorporated in the surface water and groundwater modelling approaches.
Groundwater models require information about physical properties such as the thickness of geological layers, and the hydraulic conductivity and specific storage of aquifers. As the exact values of these properties at every point and subsurface depth across the modelling domain are not known, the Assessment team used a credible range of values, which are based on various sources of data (commonly point-scale) combined with expert hydrogeological knowledge. The groundwater model was run thousands of times using a different set of plausible values for these physical properties each time. Historical observations, such as groundwater levels and changes in water movement and volume, were used to constrain and validate the model runs. Further details about the input parameter ranges used for the Galilee subregion groundwater modelling is in Table 13 of companion product 2.6.2 (Peeters et al., 2018).
The complete set of model runs produced a range or distribution of predictions (Figure 15) that are consistent with available observations and the conceptual understanding of the modelled system. The range conveys the confidence in model results, with a wide range indicating that the expected outcome is less certain, whereas a narrow range provides stronger evidence for decision making due to an increased level of confidence in the results. The distributions created from these model runs are expressed as probabilities that drawdown or a change in streamflow will exceed relevant thresholds, as there is no single ‘best’ estimate of change.
The chart on the left shows the distribution of results for drawdown, obtained from an ensemble of thousands of model runs that use many sets of parameters. These generic results are for illustrative purposes only and are not actual results from the Galilee subregion.
In this assessment, the estimates of the various hydrological response variables, such as drawdown or annual flow, are commonly presented as the 95th, 50th or 5th percentile results. These generally correspond to a 5%, 50% or 95% chance of exceeding specified thresholds. Figure 16 illustrates how the probabilistic approach applied in this BA is used to spatially segregate the subregion and its landscape classes, including criteria used to define the zone of potential hydrological change.
Throughout this product, the term ‘very likely’ is used to describe areas where there is a greater than 95% chance of something occurring, and ‘very unlikely’ is used where there is a less than 5% chance.
Figure 16 Schematic example of key areas in the landscape defined by probabilistic results
The assessment extent was divided into smaller square assessment units (see Section 3.2.4.1) and the probability distribution (Figure 15) was calculated for each. In this product, results are reported with respect to the following areas:
A. outside the zone of potential hydrological change, where hydrological changes (and hence potential impacts) are very unlikely (defined by maps showing the 95th percentile)
B. inside the zone of potential hydrological change, comprising the assessment units with at least a 5% chance of exceeding the threshold (defined by maps showing the 95th percentile). Further work is required to determine whether the hydrological changes in the zone translate into impacts for water-dependent assets and landscapes
C. with at least a 50% chance of exceeding the threshold (i.e. the assessment units where the median is greater than the threshold; defined by maps showing the 50th percentile)
D. with at least a 95% chance of exceeding the threshold (i.e. the assessment units where hydrological changes are very likely; defined by maps showing the 5th percentile).
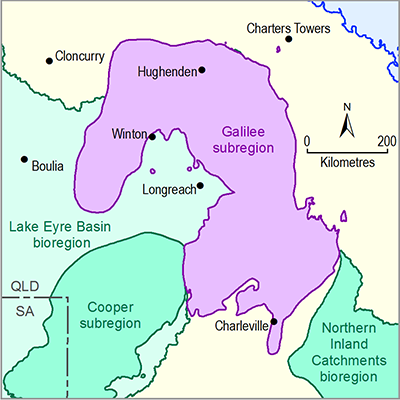
Product Finalisation date
- 3.1 Overview
- 3.2 Methods
- 3.3 Potential hydrological changes
- 3.4 Impacts on and risks to landscape classes
- 3.4.1 Overview
- 3.4.2 Landscape classes that are unlikely to be impacted
- 3.4.3 'Springs' landscape group
- 3.4.4 'Streams, GDE' landscape group
- 3.4.5 'Streams, non-GDE' landscape group
- 3.4.6 'Floodplain, terrestrial GDE' landscape group
- 3.4.7 'Non-floodplain, terrestrial GDE' landscape group
- References
- Datasets
- 3.5 Impacts on and risks to water-dependent assets
- 3.6 Commentary for coal resource developments that are not modelled
- 3.7 Conclusion
- Citation
- Acknowledgements
- Contributors to the Technical Programme
- About this technical product